Nanoscale Materials for Quantum Computing
Nanoscale Materials for Quantum Computing
Quantum computing is a rapidly advancing field that holds great promise for solving complex problems that are currently intractable for classical computers. At the heart of quantum computing are qubits, the fundamental units of quantum information. Qubits can exist in a superposition of states, allowing quantum computers to perform parallel computations and solve problems exponentially faster than classical computers. However, building a practical and scalable quantum computer requires the development of high-quality qubits that can be precisely controlled and maintained at extremely low temperatures. Nanoscale materials play a crucial role in this endeavor, offering unique properties that can be harnessed for quantum computing applications.
Introduction
Quantum computing represents a paradigm shift in computing technology, with the potential to revolutionize fields such as cryptography, drug discovery, optimization problems, and machine learning. However, building a large-scale quantum computer is a formidable challenge due to the delicate nature of qubits and their susceptibility to decoherence and errors. Nanoscale materials offer a promising avenue for overcoming these challenges and enabling the development of practical quantum computers.
Advertisement
Types of Nanoscale Materials for Quantum Computing
There are several types of nanoscale materials that have shown potential for quantum computing applications:
1. Superconducting Materials
Superconducting materials exhibit zero electrical resistance and the expulsion of magnetic fields when cooled below a certain critical temperature. They have been widely studied for their potential in creating superconducting qubits, which are among the most promising candidates for building a scalable quantum computer. Superconducting qubits can be fabricated using materials such as aluminum, niobium, and tungsten silicide, among others.
2. Semiconductor Quantum Dots
Semiconductor quantum dots are nanoscale structures that can confine and manipulate the motion of electrons, allowing for the creation of quantum bits. They offer several advantages over superconducting qubits, including longer coherence times and the ability to operate at higher temperatures. Quantum dots can be made from a variety of semiconductor materials, including gallium arsenide, silicon, and indium arsenide.
3. Topological Insulators
Topological insulators are materials that exhibit unique electronic properties, with insulating behavior in their bulk and conducting states on their surface. They have been proposed as a potential platform for creating topological qubits, which are expected to have longer coherence times and be more robust against errors compared to other qubit types. Examples of topological insulators include bismuth selenide, bismuth telluride, and antimony telluride.
4. Diamond and Nitrogen-Vacancy Centers
Diamond with nitrogen-vacancy (NV) centers has emerged as a promising material for quantum computing due to its long coherence times, high fidelity, and compatibility with existing technology. NV centers are point defects in the diamond lattice where a nitrogen atom replaces a carbon atom, and an adjacent lattice site is vacant. They can be used to create qubits that are highly sensitive to magnetic fields and can be manipulated using optical and microwave pulses.
Challenges and Opportunities
While nanoscale materials offer exciting opportunities for quantum computing, there are several challenges that need to be addressed:
1. Scalability
Building a large-scale quantum computer requires the ability to control and manipulate a large number of qubits. Scaling up the number of qubits while maintaining their coherence and minimizing errors is a significant challenge. Advances in fabrication techniques, error correction codes, and quantum algorithms are needed to overcome this challenge.
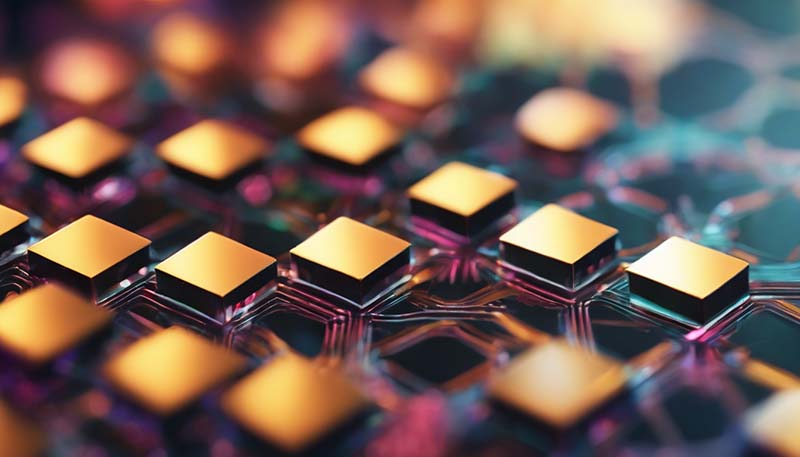
2. Material Quality and Purity
The performance of quantum devices is highly dependent on the quality and purity of the materials used. Defects, impurities, and unwanted interactions can lead to decoherence and errors in quantum computations. Developing high-quality materials with minimal defects and impurities is crucial for realizing practical quantum computers.
3. Integration with Classical Electronics
Quantum computers need to be integrated with classical electronics for control, readout, and error correction. Developing hybrid systems that combine the best of both worlds is an ongoing challenge. This requires the development of efficient interfaces and protocols for communication between quantum and classical systems.
4. Error Correction and Fault Tolerance
Quantum systems are inherently susceptible to errors due to their fragile nature. Developing robust error correction and fault-tolerant techniques is essential for building practical quantum computers. This requires the development of new quantum algorithms and error correction codes that can tolerate a certain level of errors and decoherence.
Conclusion
Nanoscale materials offer a promising avenue for the development of practical quantum computers. By leveraging their unique properties, researchers can overcome some of the key challenges in building a scalable and fault-tolerant quantum computer. While significant progress has been made in recent years, further research and development are needed to fully realize the potential of nanoscale materials for quantum computing.
As the field of quantum computing continues to advance, it is likely that new materials and technologies will emerge, offering even greater opportunities for breakthroughs in this exciting area of research. The development of practical quantum computers has the potential to revolutionize computing and solve some of the most pressing problems facing society today.